OPTICAL MEASUREMENTS OF THE LEVITATION HEIGHT OF DROPLET CLUSTERS
Following from: Droplet clusters levitating over the heated water surface
The authors developed a new method for measuring small vertical displacements of droplets near the surface of a water layer to study the behavior of the levitating droplet clusters. A brief overview of research on droplet clusters can be found in a recent paper by Fedorets and Dombrovsky (2023). The main results concerning levitating droplet clusters are detailed in the monograph (Fedorets et al., 2023b). A method for generating clusters of a given number of nearly identical droplets was found, which made it possible to consider applications of droplet clusters in microbiological experiments (Fedorets et al., 2019). Interest in such experiments is related, in particular, to the effect of significant acceleration of organic chemical reactions in small droplets (Wei et al., 2020, Zhang et al., 2021).
Some features of the behavior of droplet clusters are poorly described theoretically. Therefore, more accurate measurements of the levitation height of large water droplets would be very useful to continue the research. Photographs of the cluster along the water surface show reflections of the droplets in the water layer, which allows one to measure the height of levitation of the droplets (Fedorets et al., 2020, Zaitsev et al., 2022). The accuracy of such measurements is limited by the capabilities of optical microscopes because the image pixel size of about 0.6 μm is insufficient to measure the low levitation heights of large droplets. A method of precise measurements developed by Fedorets and Dombrovsky (2023c) is proven to be effective for these measurements.
We used the experimental setup described in (Fedorets et al., 2022, 2023a). The cluster was formed over a layer of water locally heated by a laser beam from below through an opaque substrate. Low-power sources of infrared radiation were used to stabilize the cluster. Experiments have shown that thin color halos can appear around the droplet images when a cluster is illuminated by white light. This phenomenon is observed only at low levitation heights of the cluster, and the color of the halo changes with the levitation height.
The diameter of the droplets is much larger than the wavelength of visible light, but the levitation height, understood as the distance between the lowest point of the droplet and the water layer, can be comparable to the wavelength. A very thin gap between the levitating cluster droplets and the water layer is responsible for the halo. The maximum light intensity is observed at the following values of the path difference of the light reflected from the bottom surface of the droplet and from the surface of the water layer (Born and Wolf, 2020):
\[ \Delta=2\delta=m\lambda,\quad m=1,2,3,\dots \] | (1) |
where \(\delta\) is the droplet levitation height and \(\lambda\) is the wavelength of the halo light. Equation (1) is written for the normal incidence of the white light beam.
It is known that when illuminating a transparent spherical particle, light is not only reflected and refracted, but one observes rather complicated wave effects described by the Mie scattering theory, which gives an exact analytical solution for the scattering problem (Van de Hulst, 1981, Bohren and Huffman, 1998). In particular, the surface waves propagate along the particle surface. Calculations show that in some cases this effect should be taken into account even when determining the integral characteristics of light absorption and scattering by particles (Irvine, 1965, Dombrovsky, 1974, 1996, Liou et al., 2010). In the considered problem, the light reflected from the water layer propagates from the lower surface of a droplet to its side surface and forms the observed halo. The width of the halo ring is comparable to the wavelength, but the microscope used makes the halo quite distinguishable.
The exact solution for the problem of interference of surface waves, when a droplet of water is illuminated from two sides (by a light source from above and by light passing through the droplet and reflected from the surface of the water layer), is a much more complicated task than the classical Mie problem. Note that scattering problems similar to the considered one have been solved for particles on the surface of a medium (Moreno et al., 2007), as well as for closely placed particles (Mishchenko et al., 1995). However, in the literature known to the authors, there is no solution for our specific problem. Fortunately, to interpret a periodical change of color of a droplet halo it is not necessary to solve such a complicated problem, because we are interested not in the intensity of a halo glow, but only in the periodicity of appearance of the same color when the droplet levitation height changes. Therefore, a simple formula (1) is sufficient.
It should be noted that optical interference is used to solve similar problems (Barton et al., 2012, Celestini and Kirstetter, 2012). In these studies, special schemes of measurements of the vapor gap thickness at Leidenfrost levitation of a single droplet were developed. In contrast to the mentioned papers, we do not use additional equipment and consider only the colors of the halos of the continuously descending droplets of the cluster. Therefore, we cannot determine the current absolute value of the levitation height, but the change in the halo color of droplets as they move towards the substrate water layer enables us to determine the droplet velocity.
It is also important that a droplet cluster consists of nearly identical droplets and the difference between the colors of the halos of these droplets makes it possible to estimate the difference in the levitation height of droplets of an almost flat cluster. As a result, the method of this paper provides useful information on the gradual decrease of a cluster. As for the absolute height of the droplet levitation during the process (up to the coalescence of the cluster with the water layer), it can be obtained only approximately, based on the fact that the luminous halo becomes colorless when the droplet approaches the water layer at a distance smaller than an average wavelength of visible light.
A computer code used in regular experiments to process the images of droplets was modified according to a method of (Glassner, 1989, Afanasiev et al., 2015) to analyze the halo color. The wavelength \(\lambda =\) 580 nm was assigned to white pixels, whereas the wavelength of interest was determined as the average of the twelve brightest colored pixels of the halo image. Analysis of data from four radial cross-sections of the droplet image (the orientation of the cross-sections is shown in Fig. 1(c), with three pixels each in the vicinity of the luminance maximum) allowed for smoothing of the inevitable variations in the determined halo color associated with color noise. Experiments with clusters of a small number of droplets were carried out at laser power providing surface temperature of the water layer of 55 \(\le T_\text{surf} \le\) 70°C with an error of ± 1°C. The resulting diameter of the cluster droplets varied from \(d =\) 15 μm to 60 μm.
To observe the slow growth of the droplets due to condensation, we started with an equilibrium cluster obtained by prolonged infrared heating (Dombrovsky et al., 2020). Once the infrared heating was started, the infrared heating was switched off and the droplets began to grow. Some of the results obtained are shown in Fig. 1. The halos of the equilibrium cluster droplets (at \(t \lt t_1\)) were colorless as the droplets levitated at high altitudes. The difference between the curves in Fig. 1(a) for the central and peripheral droplets increased with time, and a few seconds before the cluster coalesced with the water layer, when the levitation height was less than the emission wavelength, the halos of all droplets became colorless again. Note that the error in the measured halo wavelength and the associated droplet levitation height estimated by Fedorets et al. (2023c) is very small and does not affect the accuracy of the results obtained.
![]() |
![]() |
![]() |
Figure 1. Changes in the color of the halo around the droplets as the droplets grow and approach the water surface: (a) – wavelength of the halo of the droplets 1 and 2, (b) – average diameter of the droplets, (c) – cluster images at characteristic times, shown in panels (a) and (b) [the scale of the four images is the same, the lack of a scale bar is compensated for by the data in panel (b)]
The difference in the color change of the halos of the central and peripheral droplets in the cluster is a result of the slightly different sizes of these droplets. The central droplet surrounded by other droplets receives less vapor and therefore its size does not increase as rapidly. It is interesting that the maximum difference in diameters of droplets 1 and 2 found is less than 1 μm, whereas at the time about \(t_3\) the height of levitation of these droplets differs only by 23 nm. Interestingly, for clusters of more droplets, a regular difference in color of the halos around the droplets is observed (Fig. 2). This result further confirms the exceptionally high sensitivity of the proposed interference method to determine the levitation height of droplets. Note that with a colored halo, the entire droplet also takes on a hue of the same color. This does not help or hinder measurements but makes the image of the droplet cluster even clearer and more appealing.
![]() | ![]() |
Figure 2. Typical color halos at the droplets of stabilized clusters
Figure 3a shows the halo color curves at different temperatures of the water layer. The maximum diameter of the droplets at \(T_\text{surf} =\) 55°C was 25 μm and at \(T_\text{surf} =\) 70°C was 60 μm. Even with this significant difference in diameters, the corresponding curves in Fig. 3(a) are similar to each other. The result obtained is explained by the fact that the color of the halo does not depend on the droplet size. The change in color of the halo over time makes it possible to determine the downward velocity of the droplet (see Fig. 3b). The change in the wavelength between local maxima 1 and 3 showed that this velocity decreases approximately linearly from \(u=\) 8.4 nm/s to 5.2 nm/s as the temperature increases from \(T_\text{surf} =\) 55°C to 70°C.
![]() | ![]() |
(a) | (b) |
Figure 3. (a) – Color change of the droplet halo over time; (b) – water surface temperature's effect on the droplet's descending velocity
It is also interesting to consider the comparably fast downward motion of droplets. In a special experiment, even before the droplet sizes of the stabilized cluster were equalized, the laser heating power was drastically reduced. At the same time, the droplets practically fall down. The color change of the halos when a cluster containing droplets with diameters from \(d_1\approx\) 45 μm to \(d_1\approx\) 37 μm falls is shown in Fig. 4. At the moment of switching off the laser heating, the large droplet 1 was slightly lower than droplet 2 (its halo was already colored). The first color maximum of the large droplet was ahead of the corresponding maximum of the small droplet by about 0.04 s. In 0.12 s, the large droplet passes through two color maxima and its halo becomes colorless about 0.14 s before the cluster collapses. The color of the halo of small droplet changes several times before the collapse of the cluster.
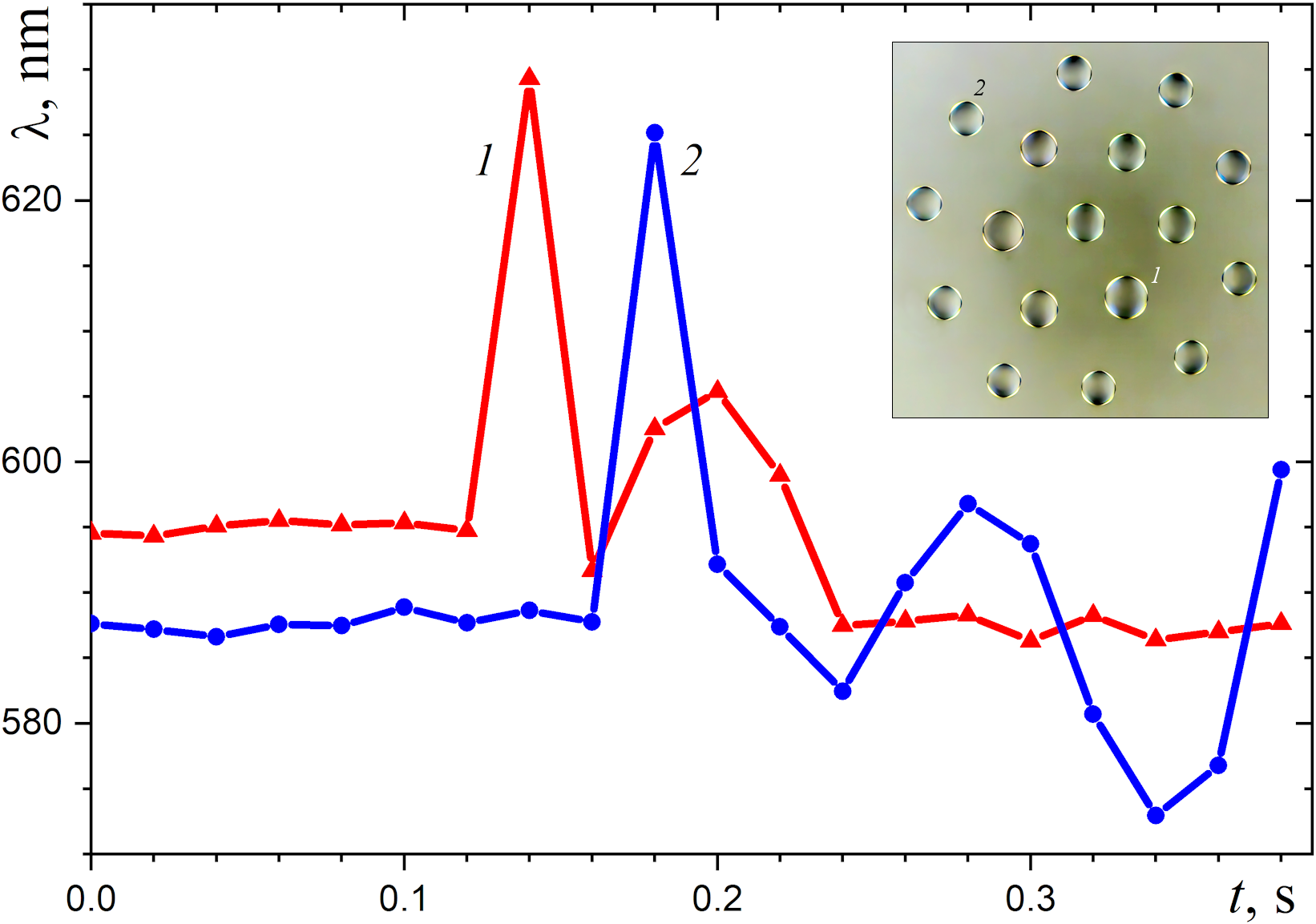
Figure 4. Changing the colored halo of the droplets 1 and 2 as the cluster falls
The droplet falling velocity is found to be approximately the same for droplets of initially different sizes: 1.8 ± 0.5 μm/s, which is about 300 times greater than the velocity of slowly growing droplets. If the minimum levitation height of a large droplet is neglected, the initial (just before switching off the laser heating) levitation height of this droplet is found to be 104 nm and the corresponding value for the second droplet is 145 nm. These values do not contradict images of a side view of the cluster, in which even at maximum microscope magnification the levitation height of the droplets does not exceed one pixel. The suggested interference method is another example of the use of optical methods in microfluidics (Choi et al., 2016, Zhou et al., 2022). The measurements carried out gave new data for the low height of levitation of droplets as well as for the downward velocity of growing microdroplets.
The motion of droplets near the water surface cannot be calculated in the usual way when the gas flow is considered in the continuum approximation and the Knudsen lifting force is ignored (Kelling and Wurm, 2009, Joung, 2011, Roy et al., 2022). The experiments with the use of the suggested optical method are expected to be important for the verification of theoretical models for the levitation of droplets at a small distance from the water surface.
REFERENCES
Afanasiev, V., Ignatenko, A., and Voloboy, A. (2015) The Simple Method of the RGB to Spectrum Conversion for Tasks of Physically Based Rendering, Scientific Visualization, 7(4): 20–26.
Barton, J.C., Sharpe, A.L., van der Veen, R.C.A., Franco, A., and Nagrl S.R. (2012) Geometry of the Vapor Layer under a Leidenfrost Drop, Phys. Rev. Lett., 109(7): 074301.
Bohren, C.F. and Huffman, D.R. (1998) Absorption and Scattering of Light by Small Particles, New York: Wiley. Born, M. and Wolf, E. (2020) Principles of Optics: 60th Anniversary Edition, Cambridge, UK: University Press.
Celestini, F. and Kirstetter, G. (2012) Effect of an Electric Field on a Leidenfrost Droplet, Soft Matter, 8(22): 5992–5995.
Choi, J.-R., Song, H., Sung, J.H., Kim, D., and Kim, K. (2016) Microfluidic Assay-Based Optical Measurement Techniques for Cell Analysis: A Review of Recent Progress, Biosens. Bioelectr., 77: 227–236.
Dombrovsky, L.A. (1974) Scattering and Absorption of Light by Hollow Spherical Particles, Izvestiya, Atmos. Ocean. Phys., 10(7): 720–727. (in Russian)
Dombrovsky, L.A. (1996) Radiation Heat Transfer in Disperse Systems, New York: Begell House.
Dombrovsky, L.A., Fedorets, A.A., Levashov, V.Yu., Kryukov, A.P., Bormashenko, E., and Nosonovsky M. (2020) Stable Cluster of Identical Water Droplets Formed under the Infrared Irradiation: Experimental Study and Theoretical Modeling, Int. J. Heat Mass Transf., 161: 120255.
Fedorets, A.A. and Dombrovsky, L.A. (2023) Levitating Droplet Clusters: From the Discovery to Potential Applications, Academia Engineering, 1: 1–6.
Fedorets, A.A., Bormashenko, E., Dombrovsky, L.A., and Nosonovsky, M. (2019) Droplet Clusters: Nature-Inspired Biological Reactors and Aerosols, Philos. Trans. Royal Soc. A, 377 (2150): 2019012.
Fedorets, A.A., Dombrovsky, L.A., Gabyshev, D.N., Bormashenko, E., and Nosonovsky, M. (2020) Effect of External Electric Field on Dynamics of Levitating Water Droplets, Int. J. Therm. Sci., 153: 106375.
Fedorets, A.A., Shcherbakov, D.V., Levashov, V.Yu., and Dombrovsky, L.A. (2022) Self-Stabilization of Droplet Clusters Levitating over Heated Salt Water, Int. J. Thermal Sci., 182: 107822.
Fedorets, A.A., Medvedev, D.N., Levashov, V.Yu., and Dombrovsky, L.A. (2023a) Stabilization of Levitating Clusters Containing Saltwater Droplets, Int. J. Thermal Sci., 188: 108222.
Fedorets, A.A., Dombrovsky, L.A., Bormashenko, E., and Nosonovsky, M. (2023b) Levitating Droplet Clusters, New York: Begell House, USA.
Fedorets, A.A., Kolmakov, E.E., and Dombrovsky, L.A. (2023c) A New Method for Precise Optical Measurements of the Sub-Micron Height of Levitation of Droplet Clusters, Particuology, 87: 173–178.
Glassner, A.S. (1989) How to Derive a Spectrum from an RGB Triplet, IEEE Comput. Graph. Appl., 9(4): 95–99.
Irvine, W.M. (1965) Light Scattering by Spherical Particles: Radiation Pressure, Asymmetry Factor, and Extinction Cross Section, J. Opt. Soc. Am., 55(1): 16–21.
Joung, J.B. (2011) Thermophoresis of a Spherical Particle: Reassessment, Clarification, and New Analysis, Aerosol Sci. Technol., 45(8): 927–948.
Kelling, T. and Wurm, G. (2009) Self-Sustained Levitation of Dust Aggregate Ensembles by Temperature-Gradient-Induced Overpressures, Phys. Rev. Lett., 103: 215502.
Liou, K.N., Takano, Y., and Yang, P. (2010). On Geometrical Optics and Surface Waves for Light Scattering by Spheres, J. Quant. Spectr. Radiat. Transf., 111(12-13): 1980–1989.
Mishchenko, M.I., Mackowski, D.W., and Travis, L.D. (1995) Scattering of Light by Bispheres with Touching and Separated Components, Appl. Optics, 34(21): 4589–4599.
Moreno, F., Saiz, J.M., and González, F. (2007) Light Scattering by Particles on Substrates. Theory and Experiments, in A.A. Maradudin (ed.), Light Scattering and Nanoscale Surface Roughness. Nanostructure Science and Technology, Chapter 12, Boston, MA: Springer, 305–340.
Roy, P.K., Legchenkova, I., Dombrovsky, L.A., Levashov, V.Yu., Binks, B.P., Shvalb, V., Shoval, S., Valtsifer, V., and Bormashenko, E. (2022) Thermophoretic Levitation of Powders at Atmospheric Pressure, Adv. Powder Technol., 33(3): 103497.
Van de Hulst, H.C. (1981) Light Scattering by Small Particles, New York: Dover Publ.
Wei, Z., Li, Y., Cooks, R.G., and Yan, X. (2020) Accelerated Reaction Kinetics in Microdroplets: Overview and Recent Developments, Ann. Rev. Phys. Chem., 71: 31–51.
Zaitsev, D.V., Kirichenko, D.P., Kabov, O.A., and Ajaev, V.S. (2021) Levitation Conditions for Condensing Droplets Over Heated Liquid Surfaces, Soft Matter, 17(17): 4623–4631.
Zhang, Z., Apsokardu, M.J., Kerecman, D.E., Achtenhagen, M., and Johnston, M.V. (2021) Reaction Kinetics of Organic Aerosol Studied by Droplet Assisted Ionization: Enhanced Reactivity in Droplets Relative to Bulk Solution, J. Am. Soc. Mass Spectrom., 32(1): 46–54.
Zhou, P., He, H., Ma, H., Wang, S., and Hu, S. (2022). A Review of Optical Imaging Technologies for Microfluidics, Micromachines, 13(2): 274.
Les références
- Afanasiev, V., Ignatenko, A., and Voloboy, A. (2015) The Simple Method of the RGB to Spectrum Conversion for Tasks of Physically Based Rendering, Scientific Visualization, 7(4): 20–26.
- Barton, J.C., Sharpe, A.L., van der Veen, R.C.A., Franco, A., and Nagrl S.R. (2012) Geometry of the Vapor Layer under a Leidenfrost Drop, Phys. Rev. Lett., 109(7): 074301.
- Bohren, C.F. and Huffman, D.R. (1998) Absorption and Scattering of Light by Small Particles, New York: Wiley. Born, M. and Wolf, E. (2020) Principles of Optics: 60th Anniversary Edition, Cambridge, UK: University Press.
- Celestini, F. and Kirstetter, G. (2012) Effect of an Electric Field on a Leidenfrost Droplet, Soft Matter, 8(22): 5992–5995.
- Choi, J.-R., Song, H., Sung, J.H., Kim, D., and Kim, K. (2016) Microfluidic Assay-Based Optical Measurement Techniques for Cell Analysis: A Review of Recent Progress, Biosens. Bioelectr., 77: 227–236.
- Dombrovsky, L.A. (1974) Scattering and Absorption of Light by Hollow Spherical Particles, Izvestiya, Atmos. Ocean. Phys., 10(7): 720–727. (in Russian)
- Dombrovsky, L.A. (1996) Radiation Heat Transfer in Disperse Systems, New York: Begell House.
- Dombrovsky, L.A., Fedorets, A.A., Levashov, V.Yu., Kryukov, A.P., Bormashenko, E., and Nosonovsky M. (2020) Stable Cluster of Identical Water Droplets Formed under the Infrared Irradiation: Experimental Study and Theoretical Modeling, Int. J. Heat Mass Transf., 161: 120255.
- Fedorets, A.A. and Dombrovsky, L.A. (2023) Levitating Droplet Clusters: From the Discovery to Potential Applications, Academia Engineering, 1: 1–6.
- Fedorets, A.A., Bormashenko, E., Dombrovsky, L.A., and Nosonovsky, M. (2019) Droplet Clusters: Nature-Inspired Biological Reactors and Aerosols, Philos. Trans. Royal Soc. A, 377 (2150): 2019012.
- Fedorets, A.A., Dombrovsky, L.A., Gabyshev, D.N., Bormashenko, E., and Nosonovsky, M. (2020) Effect of External Electric Field on Dynamics of Levitating Water Droplets, Int. J. Therm. Sci., 153: 106375.
- Fedorets, A.A., Shcherbakov, D.V., Levashov, V.Yu., and Dombrovsky, L.A. (2022) Self-Stabilization of Droplet Clusters Levitating over Heated Salt Water, Int. J. Thermal Sci., 182: 107822.
- Fedorets, A.A., Medvedev, D.N., Levashov, V.Yu., and Dombrovsky, L.A. (2023a) Stabilization of Levitating Clusters Containing Saltwater Droplets, Int. J. Thermal Sci., 188: 108222.
- Fedorets, A.A., Dombrovsky, L.A., Bormashenko, E., and Nosonovsky, M. (2023b) Levitating Droplet Clusters, New York: Begell House, USA.
- Fedorets, A.A., Kolmakov, E.E., and Dombrovsky, L.A. (2023c) A New Method for Precise Optical Measurements of the Sub-Micron Height of Levitation of Droplet Clusters, Particuology, 87: 173–178.
- Glassner, A.S. (1989) How to Derive a Spectrum from an RGB Triplet, IEEE Comput. Graph. Appl., 9(4): 95–99.
- Irvine, W.M. (1965) Light Scattering by Spherical Particles: Radiation Pressure, Asymmetry Factor, and Extinction Cross Section, J. Opt. Soc. Am., 55(1): 16–21.
- Joung, J.B. (2011) Thermophoresis of a Spherical Particle: Reassessment, Clarification, and New Analysis, Aerosol Sci. Technol., 45(8): 927–948.
- Kelling, T. and Wurm, G. (2009) Self-Sustained Levitation of Dust Aggregate Ensembles by Temperature-Gradient-Induced Overpressures, Phys. Rev. Lett., 103: 215502.
- Liou, K.N., Takano, Y., and Yang, P. (2010). On Geometrical Optics and Surface Waves for Light Scattering by Spheres, J. Quant. Spectr. Radiat. Transf., 111(12-13): 1980–1989.
- Mishchenko, M.I., Mackowski, D.W., and Travis, L.D. (1995) Scattering of Light by Bispheres with Touching and Separated Components, Appl. Optics, 34(21): 4589–4599.
- Moreno, F., Saiz, J.M., and González, F. (2007) Light Scattering by Particles on Substrates. Theory and Experiments, in A.A. Maradudin (ed.), Light Scattering and Nanoscale Surface Roughness. Nanostructure Science and Technology, Chapter 12, Boston, MA: Springer, 305–340.
- Roy, P.K., Legchenkova, I., Dombrovsky, L.A., Levashov, V.Yu., Binks, B.P., Shvalb, V., Shoval, S., Valtsifer, V., and Bormashenko, E. (2022) Thermophoretic Levitation of Powders at Atmospheric Pressure, Adv. Powder Technol., 33(3): 103497.
- Van de Hulst, H.C. (1981) Light Scattering by Small Particles, New York: Dover Publ.
- Wei, Z., Li, Y., Cooks, R.G., and Yan, X. (2020) Accelerated Reaction Kinetics in Microdroplets: Overview and Recent Developments, Ann. Rev. Phys. Chem., 71: 31–51.
- Zaitsev, D.V., Kirichenko, D.P., Kabov, O.A., and Ajaev, V.S. (2021) Levitation Conditions for Condensing Droplets Over Heated Liquid Surfaces, Soft Matter, 17(17): 4623–4631.
- Zhang, Z., Apsokardu, M.J., Kerecman, D.E., Achtenhagen, M., and Johnston, M.V. (2021) Reaction Kinetics of Organic Aerosol Studied by Droplet Assisted Ionization: Enhanced Reactivity in Droplets Relative to Bulk Solution, J. Am. Soc. Mass Spectrom., 32(1): 46–54.
- Zhou, P., He, H., Ma, H., Wang, S., and Hu, S. (2022). A Review of Optical Imaging Technologies for Microfluidics, Micromachines, 13(2): 274.