Deep Geothermal Heat
Undoubtedly, the greatest prospects in the future geothermal energy are associated with the development of deep geothermal heat, which is accumulated in hot dry rocks at technically accessible depths from 3 to 10 km (Glassley, 2015; Massachusetts Institute of Technology, 2006). Apparently, the idea of extracting geothermal energy from solid hot rocks was first expressed by K.E. Tsiolkovsky in 1897 and described in more detail in 1914 (Alekseenko et al., 2016). Tsiolkovsky considered the heating of water due to heat exchange with rocks having a temperature of 120°C at a depth of more than 4 km, and its circulation in two vertical channels. About the same time, the Hell Fire Exploration Project was developed, which was initiated in 1904 by Charles Parsons, the famous English engineer, and inventor of the steam turbine. The project provided for building a zigzag mine at a depth of 19 km to the high-temperature horizons of the subsurface. The first flow diagram of the geothermal circulation system (GCS) was proposed by academician V.A. Obruchev in 1920 in his novel “Thermal Mine”. The first geothermal circulation system, extracting heat from layers with natural permeability was built in Paris in 1963. By 1985, 64 GCSs with a total thermal capacity of 450 MW provided heat to 154 thousand urban apartments.
The schematic diagram of the deep geothermal heat utilization is shown in Fig. 1. Cold water enters the reservoir, situated in permeable hot dry rocks through the injection well, heats up, comes out to Earth surface through production wells, and then enters the geothermal power plant (GPP) in the form of hot water or steam. From the GPP, the water returns to the injection well again, thereby creating a closed circulation system. Since natural permeability at great depths is rare due to the predomination of solid granite and basalt rocks, a need arises to create an artificial permeable reservoir. In 1970, the Los Alamos National Laboratory (USA) proposed a GCS with an artificial collector representing vertical cracks created by hydraulic fracturing in a monolith. In hydraulic fracturing, the bottom-hole pressure should be 1.8–2.5 times higher than the hydrostatic pressure. To prevent cracks, produced by hydraulic fracturing, from closing when the pressure decreases, they are fixed with a proppant, which is usually quartz sand with a fraction of 0.5–0.8 mm, pumped into the cracks by a viscous liquid. Similar hydraulic fracturing is also used in oil production, however, the water consumption in geothermal wells should be ten times greater than in oil production. This project was called Hot Dry Rock (HDR). It was implemented in the town of Fenton Hill in the Valles Caldera in New Mexico in 1973–1996. At the first stage of the project, two wells were successfully drilled to a depth of about 2,600 m. The wells were communicated through a rupture field in an artificially created reservoir and were capable of producing hot liquid at a temperature of 135–140°C with a flow rate ranging from 7 to 16 kg/s. A binary plant with a capacity of 60 kW was installed as a means of generating electricity from hot water circulating through the loop. During the second stage, two more wells were drilled at a distance of 50 m from each other. The deeper well extended to 4,390 m, where the temperature reached 327°C. Unfortunately, two new wells were not communicated, and it took a lot of effort to close the hydraulic loop. Thus, certain challenging problems of creating GCSs were identified. In particular, the standard method of hydraulic fracturing gave an insufficient number of cracks to achieve necessary permeability and good heat exchange. Therefore, further projects were oriented toward creating extensive reservoirs with many cracks arising by stimulating natural defects of the rock. Such projects are called Enhanced Geothermal Systems (EGS) (Glassley, 2015; Massachusetts Institute of Technology, 2006). The difference between HDR and EGS is shown in Fig. 2. In total, about twenty experimental HDR or EGS systems were implemented, which confirmed the technological ability to extract deep heat from hot dry rocks at depths up to 5.1 km.
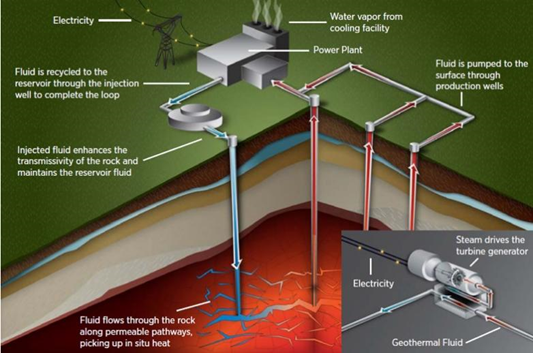
Figure 1. Deep heat utilization diagram
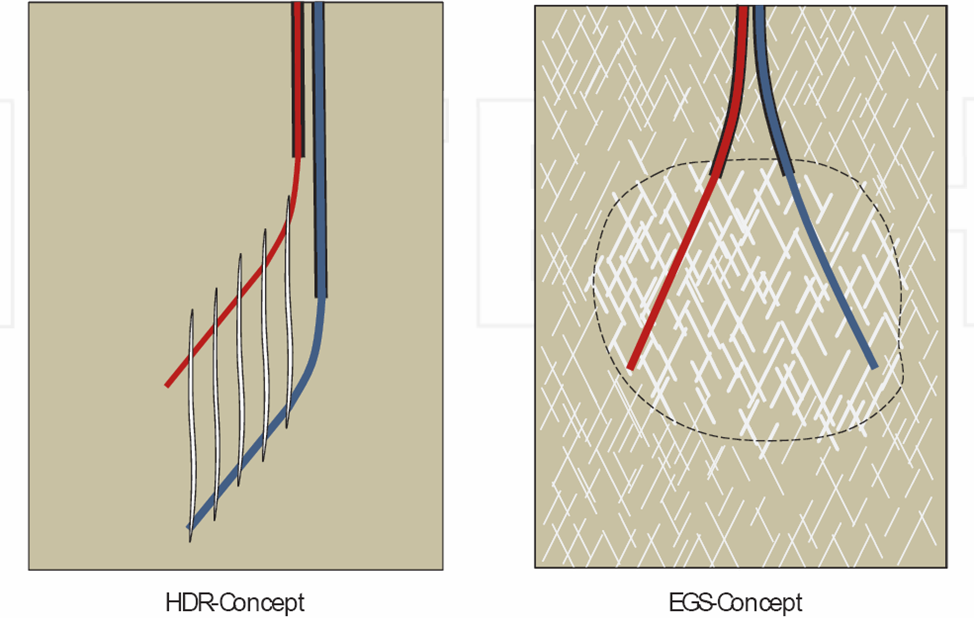
Figure 2. Types of circulation systems: (left) Hot Dry Rock (HDR); (right) Enhanced Geothermal Systems (EGS)
Here we have to make an important remark about terminology. Department of Energy US offers the following: «Although many discussions regarding EGS are commonly referenced to relatively deep environments (∼ > 6 km), enhancement of geothermal systems using engineering methods can be accomplished under any set of conditions. Hence, the phrase “enhanced geothermal systems” should not be restricted to only deep geothermal systems—any system that has been engineered by technological means in such a way that improved permeability or fluid mass flow can be considered an enhanced geothermal system». Hence, HDR is a special case of EGS.
Recently, the problems on terminology are discussed more actively. In particular, in Çiçek (2020) a number of concepts are discussed, viz., Enhanced Geothermal Systems (EGS), Engineered Geothermal Systems (EGS), Hot Wet Rock (HWR), Deep Geothermal Energy (DGE), Petrothermal Systems (PS), Hot Fractured Rock (HFR), Hot Sedimentary Aquifers (HSA), Unconventional Geothermal Systems (UGS), etc. As a result of analysis it is suggested to replace EGS by a more general term Unconventional Geothermal Systems, (UGS). The Unconventional Geothermal Systems (UGS) term can be defined as “any hot rock without commercial natural geothermal fluid production after conventional well completion works.” However, this definition has rather commercial, than physical character. A more physical approach is suggested in Breede et al. (2015): “This study recommends re-introducing three known definitions such as Petrothermal, Hydrothermal, as well as HSA, and abandoning the ambiguous terminology such as EGS.” Here an additional concept—HSA (Hot Sedimentary Aquifers), which has the features of both hydrothermal and petrothermal systems, is introduced. Nevertheless, since conventional terms have not been accepted as yet, we will mainly use the EGS term with additional explanations of geothermal system details.
The characteristics of the major EGS projects (Breede et al., 2015; Sigfusson and Uihlein, 2015) are shown in Table 1. Among the participating countries are the USA, Japan, Great Britain, France, Germany, and Australia. Even small commercial stations were operating, such as Desert Peak Demonstration Project in Nevada with a capacity of 1.7 MW (since 2013) and Landau in Germany.
TABLE 1: Parameters of circulation systems
Location | System | Years | Depth (m) | Temperature (°C) | Flow Rate (l/s) | Power (MW) | Type | Status |
---|---|---|---|---|---|---|---|---|
Fenton Hill, New Mexico | Petrothermal | 1974–1993 | 2932–4390 | 180–327 | 10.6–18.5 | 0.06 | Research facility | Concluded |
Rosemanowes, United Kingdom | Petrothermal | 1977–1992 | 2000–2600 | 79–100 | 4–15 | — | Research facility | Concluded |
La Mayet, France | Petrothermal | 1978–1987 | 200–800 | 22 | 5.2 | — | Research facility | Concluded |
Hijiori, Japan | Petrothermal | 1985–2002 | 1800; 2200 | 250; 270 | 17 | 0.13 | Research facility | Concluded |
Soultz-sous-Forêts, France | Petrothermal | 1984–present | 3600–5000 | 165 | 30 | 1.0 | Research facility | Ongoing |
Landau, Germany | HSA | 2003–present | 3170–3300 | 159 | 70–80 | 3.0 | Commercial plant | Ongoing |
Cooper Basin, Australia | Petrothermal | 2003–2013 | 3700–4911 | 242–278 | 35 per well | 1.0 Habanero |
Pilot plant | Abandoned |
Newberry, Oregon | Petrothermal | 2010–present | 3075 | 315 | 25 | 35 possible | Research facility | Ongoing |
Desert Peak, Nevada | HSA | 2002–2013 | 1000 | 210 | 32–101 | 1.7 | Commercial plant | Concluded |
The following conclusions can be drawn based on the analysis of Enhanced Geothermal Systems in relation to the case of deep dry rocks (or Petrothermal Systems). Typical EGS must meet the following requirements: the well depth should be more than 3 km; the generated power—more than 3–10 MW; the temperature in the reservoir—more than 250°C; water flow rate—50–100 kg/s; the service life—more than 25 years; the distance between wells—0.5–2 km; and the volume of the underground permeable reservoir—0.1–0.3 km3.
There is a range of technical problems:
- High drilling cost (growing exponentially with depth);
- Creating an underground reservoir with sufficient permeability;
- Uncertainty and short lifetime of a paired wells;
- Temperature drop over time (decrease in EGS efficiency);
- Low efficiency of heat extraction amounting to 1–5% of the available reserve;
- The need for water for EGS and its loss in the system;
- Creating a closed circulation system (drilling of subsequent wells);
- Induced seismicity;
- Corrosion of equipment due to HCl, H2S, and carbonates.
The first two fundamental problems are the most important. Drilling wells is very expensive for any industry. More than 60% of the total capital expenditure for EGS may fall on drilling. Thus, the price of drilling a 10 km deep well is estimated at 15–30 million dollars. Typical parameters of a geothermal well are as follows: diameter at the well inlet – 500 mm, at the well bottom – 150 mm, drill penetration rate – up to 10 m/h. For monitoring purposes, so-called “microholes” with an inlet diameter of 120 mm, and 50 mm at the bottom, and a very high drill penetration rate of up to 60 m/h (1.5 km/day) have recently been applied. Placing geophones along the “microhole” allows conducting tomographic monitoring which is fundamentally important for decision-making on the construction of EGS. Thus, the search for new technologies for high-speed and cheap drilling is of fundamental importance for many industries. As a result of the implementation of the Department of Energy (DOE) Geothermal Energy Program, various technologies for drilling “microholes” were developed and tested in 2005–2007. These technologies include resonant drilling, high-pressure fluid enhanced cutting, and high-speed drilling at 5000 rpm. Laser and microwave drilling methods have also been considered but have not yet found application. Another technology could be based on using plasma to soften basalt rocks before drilling, but this approach is currently at the stage of scientific research.
Attention should be drawn to the low efficiency of heat extraction (just 1–5% of the available reserve) and the drop in the temperature of the heat carrier over time, which leads to a decrease in the efficiency of EGS. In this regard, the analysis of heat transfer in EGS is very important. Figure 3 illustrates the results of calculating the parameters of a GCS with several fractures for model conditions (Alekseenko et al., 2016), which can be used for service evaluations. The following are shown in the Figure: (a) the heat carrier temperature at the outlet of the GCS with the number of cracks n and the flow rate of 43 m3/h; (b) the dependence of the GCS service life on the flow rate of the heat carrier at n = 5; (c) the dependence of the GCS capacity on time t at n = 5 and the total flow rate of the coolant, equal to 43 m3/h. It can be seen that the electrical power varies over time. Three phases of power change at a constant water velocity were identified in Jeanloz et al. (2013): 1 – constant power; 2 – power decrease over time according to the law of t–1/2; 3 – power decrease according to the law of t–1. To increase efficiency, a strategy is proposed: at stage 3, reduce the speed of water pumping in order to reduce the power drop to the law of stage 2.
![]() | ![]() | ![]() |
(a) | (b) | (c) |
Figure 3. Parameters of the GCS under different conditions: (a) the temperature of the heat carrier at the outlet of the GCS with the number of cracks n and the flow rate of 43 m3/hour; solid line – numerical computation, dashed line – analytical calculation; (b) the dependence of the GCS service life on the heat carrier flow rate at n = 5; (c) the dependence of the GCS capacity on time at n = 5 and the total flow rate of heat carrier of 43 m3/hour
The following recommendations can be suggested to further develop EGS:
- Creating integration programs for laboratory and field research, including experimental and theoretical studies, as well as numerical simulations to study heat transfer, permeability, mechanical properties of rocks, etc.
- Applying and developing modern geophysical diagnostic methods, such as deep micro-drilling with sensors installed along the depth; visualizing seismic data; carrying out electromagnetic monitoring; and applying tracer methods.
- Interacting with traditional geothermal systems, especially in the field of power equipment (GPPs driven by dry steam and steam-water mixture, binary GPPs, and heat pumps), as well as with the oil and gas industry (exploration, drilling).
Let's highlight the undeniable advantages of deep geothermal heat, which are not peculiar in other energy sources:
- Continuous energy production;
- Energy can be produced anywhere on Earth;
- The renewable energy source (RES);
- No environmental impacts;
- No emission of gases, including CO2;
- No need to store primary energy;
- Energy production does not need large land areas;
- An inexhaustible source of energy;
- Cheap energy source.
Below are a few more facts to confirm the advantage of deep geothermal heat. Using the example of the USA, it is shown in Massachusetts Institute of Technology (2006) that deep geothermal heat just at depths up to 10 km contains 130 thousand times more energy than the annual energy consumption of the USA. With an extraction efficiency of 1.5%, this energy source will last for two thousand years, and if applying advanced technologies – for 20,000 years, even without taking into account its renewal. This leads to a global conclusion: deep geothermal heat is enough to provide humanity with energy forever (taking into account the finite time of the existence of civilizations). As for prices, the same estimates (Massachusetts Institute of Technology, 2006) show that by 2030, it is possible to achieve a levelized cost of deep geothermal energy (LCOE) of up to 6 ¢/kWh, which is at the level of the lowest indicators for the energy sector.
Although the above information concerns mainly scientific research and experimental setups, nevertheless there is no doubt about the reality of the large-scale application of deep heat in practice. It is enough to cite the recently published impressive plans of the USA, the leader in this area, stated in the program “GeoVision: Harnessing the Heat Beneath Our Feet, 2019” [https://openei.org/apps/geovision]. By 2050, it is planned to reach 60 GWe due to deep heat. This will amount to 3.7% of the total installed capacity of the USA in 2050, and in terms of total energy production – 8.5% of all electricity generation in the country (20.4% of energy, produced by all RES). Moreover, ambitious targets concern direct use of heat – 320 GW instead of today's 0.1 GW. The stated targets are confirmed financially – the federal budget for geothermal research in 2020 amounted to an impressive figure of $110 million, of which $69 million were allocated for deep geothermal heat.
In 2023, the U.S. Department of Energy announced a new initiative, the so-called Enhanced Geothermal Shot™. According to this initiative, much higher indices are planned by 2050—90 GWe in power and by about 4.5 ¢/kWh in levelized cost of energy (LCOE).
There exist others, less known and only experimentally tested, means of creating a closed circulating system which should also be classified as Enhanced Geothermal System (EGS). They are called Advanced Geothermal Systems (AGS). Instead of an underground permeable reservoir, different types of systems of interconnected channels are produced. Eavor Technologies Inc. (Canada) has developed the Eavor-Loop™ technology (www.eavor.com) that is based on the connection of two vertical wells with many horizontal multilateral wellbores creating a closed sealed radiator-like system (Fig. 4). The Eavor proprietary working fluid is used. A full-scale prototype of the Eavor technology suite, 2.4-km deep and 2-km long, is located near Rocky Mountain House, Alberta, Canada. It was manufactured and launched in 2019. Water circulates in it for more than a year without pumping—a thermosyphon principle is realized. Its prime cost is estimated as 5 ¢/kWh.
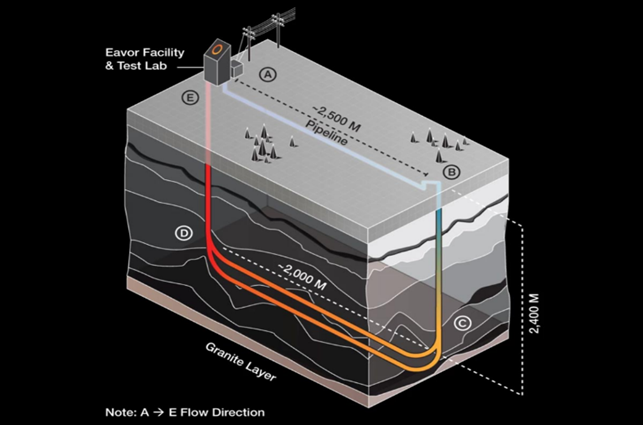
Figure 4. Technology of creating Eavor-Loop™ circulating system
A number of proposals on creation of a closed circulating system with only one vertical well of the tube-in-tube type is available. This method presupposes the use of a set of heat pipes that take heat from the environment and transfer it to the well. This technology has not been fully developed as yet and is rather complex in terms of engineering implementation.
An unusual project, related to an attempt to use the heat of magma near active volcanoes is being implemented in Iceland under the name of Iceland Deep Drilling Project (IDDP) (IEA, 2020). If successful, this project could open a new era in the development of geothermal energy. The main goal is to find out whether it is economically feasible to extract energy and chemicals from hydrothermal systems under supercritical conditions. To do this, it is necessary to penetrate an extensive reservoir with supercritical water with a temperature t = 400–600°C, located at a depth of 4–5 km. At the first well in Krafla with a depth of 2.1 km, superheated steam (with a high content of HCl, SiO2, and S) was extracted at a pressure of 140 bar and a temperature of 450°C with a potential capacity of 40 MW(e). Thus, the world's first Magma-EGS system (IDDP-1) was launched. However, the water reservoir was not very large, and therefore, a second IDDP-2 well was drilled in Reykjanes in 2017. As a result, supercritical conditions were achieved at a depth of 4,659 m at a temperature of 427°C and a pressure of 340 bar. According to a plan, a third IDDP-3 well will be drilled in the Hengill area. The possibilities of using magma heat and the analysis of volcanic activity are discussed in Dobson et al. (2017) and Koulakov et al. (2021).
REFERENCES
Glassley, W.E. (2015) Geothermal Energy: Renewable Energy and the Environment, 2nd ed., Energy and the Environment, Abbas Ghassemi, Series Editor, CRC Press, Taylor & Francis Group, 410 p.
The Future of Geothermal Energy in the 21st Century Impact of Enhanced Geothermal Systems (EGS) on the United States. Report. (2006), Massachusetts Institute of Technology. 358 p.
Alekseenko, S.V., Borodulin, V.Yu., Gnatus, N.A., Nizovtsev, M.I., Smirnova, N.N. (2016) Problems and Outlooks for Petrothermal Power Engineering (Review), Thermophysics and Aeromechanics, 23(1), pp. 1–16.
Çiçek, A. (2020) The Electric Power Production Targeted Unconventional Geothermal Systems (UGS), Some Conceptual Designs and Their Thermodynamics Classification, Bulletin of the Mineral Research and Exploration, 163, 211–228. https://doi.org/10.19111/bulletinofmre.660706.
Breede, K., Dzebisashvili, K., and Falcone, G. (2015) Overcoming Challenges in the Classification of Deep Geothermal Potential, Geoth. Energ. Sci., 3, 19–39. https://www.geoth-energ-sci.net/3/19/2015/. DOI: 10.5194/gtes-3-19-2015
Sigfusson, B., Uihlein, A.; 2015 JRC Geothermal Energy Status Report, EUR 27623 EN. DOI: 10.2790/757652
Jeanloz, R., Stone, H. et al. (2013) Enhanced Geothermal Systems [Report], Washington, DC 20585: US Department of Energy (DOE), Energy Efficiency and Renewable Energy.
Annual Report, 2019, IEA Geothermal, July 2020.
Dobson, P., Asanuma, H., Huenges, E., Poletto, F., Reinsch, T., et al. (2017). Supercritical Geothermal Systems–A Review of Past Studies and Ongoing Research Activities, 42nd Workshop on Geothermal Reservoir Engineering, Feb. 2017, Stanford, CA, United States. hal-01497951.
Koulakov, I., Komzeleva, V., Smirnov, S.Z., and Bortnikova, S.B. (2021) Magma-Fluid Interactions beneath Akutan Volcano in the Aleutian Arc Based on the Results of Local Earthquake Tomography, Journal of Geophysical Research, Solid Earth, 126, e2020JB021192. DOI: 10.1029/2020JB021192
Les références
- Glassley, W.E. (2015) Geothermal Energy: Renewable Energy and the Environment, 2nd ed., Energy and the Environment, Abbas Ghassemi, Series Editor, CRC Press, Taylor & Francis Group, 410 p.
- The Future of Geothermal Energy in the 21st Century Impact of Enhanced Geothermal Systems (EGS) on the United States. Report. (2006), Massachusetts Institute of Technology. 358 p.
- Alekseenko, S.V., Borodulin, V.Yu., Gnatus, N.A., Nizovtsev, M.I., Smirnova, N.N. (2016) Problems and Outlooks for Petrothermal Power Engineering (Review), Thermophysics and Aeromechanics, 23(1), pp. 1–16.
- Çiçek, A. (2020) The Electric Power Production Targeted Unconventional Geothermal Systems (UGS), Some Conceptual Designs and Their Thermodynamics Classification, Bulletin of the Mineral Research and Exploration, 163, 211–228. https://doi.org/10.19111/bulletinofmre.660706.
- Breede, K., Dzebisashvili, K., and Falcone, G. (2015) Overcoming Challenges in the Classification of Deep Geothermal Potential, Geoth. Energ. Sci., 3, 19–39. https://www.geoth-energ-sci.net/3/19/2015/. DOI: 10.5194/gtes-3-19-2015
- Sigfusson, B., Uihlein, A.; 2015 JRC Geothermal Energy Status Report, EUR 27623 EN. DOI: 10.2790/757652
- Jeanloz, R., Stone, H. et al. (2013) Enhanced Geothermal Systems [Report], Washington, DC 20585: US Department of Energy (DOE), Energy Efficiency and Renewable Energy.
- Annual Report, 2019, IEA Geothermal, July 2020.
- Dobson, P., Asanuma, H., Huenges, E., Poletto, F., Reinsch, T., et al. (2017). Supercritical Geothermal Systems–A Review of Past Studies and Ongoing Research Activities, 42nd Workshop on Geothermal Reservoir Engineering, Feb. 2017, Stanford, CA, United States. hal-01497951.
- Koulakov, I., Komzeleva, V., Smirnov, S.Z., and Bortnikova, S.B. (2021) Magma-Fluid Interactions beneath Akutan Volcano in the Aleutian Arc Based on the Results of Local Earthquake Tomography, Journal of Geophysical Research, Solid Earth, 126, e2020JB021192. DOI: 10.1029/2020JB021192