Geothermal Heat Utilization Methods
In the case of dry steam, the flow diagram for generating electricity is the simplest [Fig. 1(a)], and the main problems are related to the corrosion of equipment (Alkhasov, 2008; DiPippo, 2005). For a liquid or two-phase medium, the technical solutions for organizing a geofluid thermal energy conversion system into electricity are largely determined by medium temperature. In this regard, geofluids can be conveniently divided into five groups. Table 1 shows the most promising energy conversion systems for geofluids of different temperatures (Massachusetts Institute of Technology, 2006), although the classification may differ markedly from one author to another. Figure 2 shows the installed capacity of geothermal power plants of various types in the world and the USA.
![]() | ![]() |
(a) | (b) |
Figure 1. Dry steam (a) and flash steam (b) power stations
TABLE 1: Methods of geothermal heat recovery
Geofluid Temperature,°C | Energy-Converting Systems | Typical Application | Working Fluid | Cooling System |
---|---|---|---|---|
100 | basic binary | the water of gas and oil wells | R-134a | water (evaporative condenser) |
150 | binarywith recovery | the water of gas and oil wells | isobutane | air |
200 | binary or single-flash rapid boiling | enhanced geothermal systems | isobutane or geofluid | air or water |
250 | double-flash rapid boiling | enhanced geothermal systems | geofluid | water |
400 | single-flash or three-stage expansion | supercritical enhanced geothermal systems | geofluid | water |
![]() | ![]() |
(a) | (b) |
Figure 2. The installed capacity of geothermal power plants in the world (a) and the USA (b)
Binary systems-based GPPs are successfully used for low- and medium-temperature geofluids and will be discussed in detail below. At a geofluid temperature of 200°C and above, it is possible to use relatively simple single-flash rapid boiling stations. The schematic diagram of such a station is shown in Fig. 1(b). The process of rapid boiling-up consists in transforming a liquid under pressure into a two-phase liquid-vapor medium by sharply reducing the pressure below the saturation pressure. Geofluid flows from a geothermal well through a system of valves tangentially into a cyclone separator, where the liquid fraction is separated from steam. The liquid is returned to the underground loop through an injection well. Steam passes to the inlet of the turbine that drives the electric generator. Turbines must be made of corrosion-resistant steel due to the presence of gases, such as hydrogen sulfide in the steam. The pressure of saturated steam at the turbine inlet is 5–10 bar, which is significantly different from the conditions that occur at organic fuel-fired thermal power plants using highly superheated steam. This results in the emergence of a large amount of liquid in the turbine, which contributes to erosion on the turbine blades. Turbines for single-flash geothermal plants are usually manufactured with a capacity of 25–55 MW. After the turbine, the steam condenses in a surface condenser. Gases, such as CO2 and H2S do not condense in the surface condenser, increase the overall pressure, and reduce the operating efficiency of the turbine. They can be removed using steam injectors and vacuum pumps. Single-flash technologies are the dominant type in the global geothermal energy industry [see Fig. 2(a)].
At a geofluid temperature above 250°C, a rapid boiling up double-flash geothermal power plant is used (DiPippo, 2005). In contrast to the single-flash flow diagram, an instantaneous evaporation unit and a low-pressure steam line connecting the latter with the turbine are added to the double-flash flow diagram. The turbine has two inputs – for low pressure and higher pressure vapor. In such a flow diagram, two different turbines can be used. For a relatively high-power geothermal plant (55 MW and above), employing turbines with a double steam flow is considered a successful solution that allows significantly reducing the height of the outlet turbine blades. It is such stations that dominate among the flash-based technologies in the USA [see Fig. 2(b)]. For the case where the geofluid has supercritical parameters, that is, a temperature above 374°C and pressure above 22 MPa, it is promising to use a three-stage expansion flow diagram in a GPP (Massachusetts Institute of Technology, 2006). This flow diagram differs from the conventional double-flash flow diagram by adding a back pressure turbine at the inlet. Such a turbine is similar to the supercritical double-heating turbines, typical of fossil-fuel-fired power plants. It should be noted that this flow diagram requires a certain composition of steam to avoid problems with erosion of the turbine blades.
The schematic diagram of the basic binary GPP is shown in Fig. 3(a) (DiPippo, 2005). Geofluid is pumped into the evaporating heat exchanger from a geothermal well through the sand filter. In this device, the energy of the geofluid is transferred to a low-boiling working fluid. The vapors of the working fluid rotate the turbine with an electric generator. The waste steam enters the condenser, where it is converted into a liquid, which is pumped into the heater. The number of binary stations is growing most rapidly in comparison with other types of geothermal stations (see Fig. 2). They are the most promising not only for geothermal energy but also for other industries where low-potential heat recovery is required since energy can be generated even at temperatures of the order of 70°C (Bertani, 2012). The main manufacturer of binary stations equipment is ORMAT [http://www.ormat.com/].
![]() | ![]() |
(a) | (b) |
Figure 3. Binary GPP: (a) schematic diagram; (b) generator hall of the binary Paratunskaya GPP (Kamchatka, Russia, 1967–1974)
In the simplest and most common version of a binary power unit, the usual Rankine cycle for overheating steam is used, whose T-S diagram is shown in Fig. 4(d). Here, 1-2-3-4-5-6-7 is the working fluid cycle: 1-2 corresponds to compression in the pump; 2-3 – heating in the economizer; 3-4 – evaporation at a constant temperature; 4-5 – overheating in the superheater; 5-6 – expansion in the turbine; 6-7-1 – cooling and condensation in the condenser. The TA–TB line shows the temperature change of the separated liquid, and ΔT means the minimum temperature difference between the separator and the working fluid. The world's first geothermal binary power unit was implemented according to this diagram at the Paratunskaya Geothermal Experimental Industrial Power Plant in Kamchatka, Russia in 1967 (DiPippo, 2005; Ogurechnikov, 2014). The binary unit with a power of 750 kW was operating using freon R12; the temperature of the separation liquid was 80°C [Fig. 3(b)]. Operational research was carried out in the period from 1967 to 1974 by the specialists of the Institute of Thermophysics, Siberian Branch of the USSR Academy of Sciences. Subsequently, new diagrams were developed and a wide variety of working fluids were used (DiPippo, 2005; Bao and Zhao, 2013; Wang et al., 2013, etc.). Despite a fairly large number of publications on binary cycles, there is practically no information on the experience of commercial use of binary power units. Most of the works are devoted to theoretical studies of binary cycles. The greatest attention is paid to the selection of working fluid for the Organic Rankine Cycle (ORC), as the Rankine cycle is commonly called when using non-aqueous working fluids (Wang et al., 2013; Alekseenko and Ogurechnikov, 2019, 2021; Braimakis et al., 2015, etc.).
However, today the problem of choosing working fluids remains open for the following reasons. There is a huge number (more than a hundred) of potential liquids which can be used as working fluids in the ORC. Implementing ORC requires a wide range of conditions, primarily associated with the temperature of the heat source. When choosing a working fluid, various selection criteria are applied, including thermal efficiency, exergetic efficiency, heat utilization factor, heat exchanger area per unit of power, and efficiency. In recent years, environmental safety has become one of the mandatory requirements, first of all, low ODP (ozone safety) and low GWP (weak greenhouse effect). Depending on the criterion, the working fluids under consideration were ranked completely differently each time. Among the most important characteristics of working fluid (WF) is the saturation curve. Three types of curves in the temperature-entropy (T-S) coordinates are distinguished: “dry” liquid with a positive slope of the curve on the right; “wet” liquid with a negative slope, dS/dT < 0, and isentropic liquid with an infinitely large slope, dT/dS → ∞ [see Figs. 4( a)–(c)]. In the case of a “wet” liquid, the steam in front of the turbine should be overheated to such an extent as to avoid unacceptable droplet formation in the turbine. For this purpose, an expensive superheater is used [see process 4-5 in Fig. 4(d)]. It is recommended to maintain the steam dryness value at the turbine outlet at a level above 85% (Bao and Zhao, 2013). Isentropic and “dry” liquids do not require overheating; therefore they are more preferable for ORC. However, if the liquid is “too dry”, the steam leaves the turbine significantly overheated, so additional heat removal is necessary before the condensation process begins [see process 6-7 in Fig. 4(d)]. A possible solution to this problem is using heat recovery in the cycle (Alekseenko and Ogurechnikov, 2021; Franco, 2011). As for the steam overheating in front of the turbine, it has little effect on thermal efficiency. Therefore, for organic liquids, there is no need for excessive overheating (Bao and Zhao, 2013). Below are examples of various working fluids according to the classification, given in Wang et al. (2013): R21, R22, R32, R134a, R152a, R143a are “wet” liquids; R123, R124, R142b, isobutane, R141b are isentropic liquids; and R227ea, R236fa, R245fa, R600, isopentane are “dry” liquids.
![]() | ![]() | ![]() |
|
(a) | (b) | (c) | |
![]() | ![]() | ||
(d) | (e) |
Figure 4. Organic Rankine Cycle (ORC): (a), (b), (c) – examples of saturation curves; (d) the steam turbine cycle in the T-S diagram and the heat carrier temperature change; (e) T-S diagram of a supercritical cycle
Although there is no single criterion for choosing a working fluid in the ORC, nevertheless, several fairly general recommendations are available (Alkhasov, 2008; Bao and Zhao, 2013; DiPippo, 2005, 2015; Wang et al., 2013). A single working fluid can't meet all the requirements. Therefore, as noted above, dozens of substances are considered and analyzed as potential candidates for ORC working fluids, depending on the required conditions. A summary diagram on the optimal choice of working fluids is shown in Fig. 5 depending on the temperature of the heat source (Wang et al., 2013). Isobutane and R142b are considered among the most promising working fluids (Alkhasov, 2008). However, isobutane is a highly flammable substance, while R142b, when interacting with water, forms halogen acids, which have a corrosive effect on structural materials. As a consequence of these selection difficulties, only a few substances are used for commercial purposes, namely, R134a, R245fa, N-pentane, isobutane, isopentane, as well as some mixtures, in particular, isobutane-isopentane mixture. It should be noted that a special area has been formed in the world, associated with using non-azeotropic mixtures, whose boiling and condensation occur at variable temperatures. As a result, temperature heads and irreversible losses are reduced and the efficiency of power plants is increased (DiPippo, 2005, 2015). The most striking example is the so-called Kalina cycle, which uses a water-ammonia solution. This cycle has been successfully tested in several pilot projects. It should be noted that ammonia looks particularly promising because it has been mastered on a global scale, in addition, it is the best way to store hydrogen and it is an efficient fuel for fuel cells. By comparing pure substances and their mixtures for subcritical and supercritical Rankine cycles, it is shown that non-azeotropic mixtures (for example, R134a/R32) demonstrate the best efficiency in supercritical conditions (Braimakis et al., 2015).
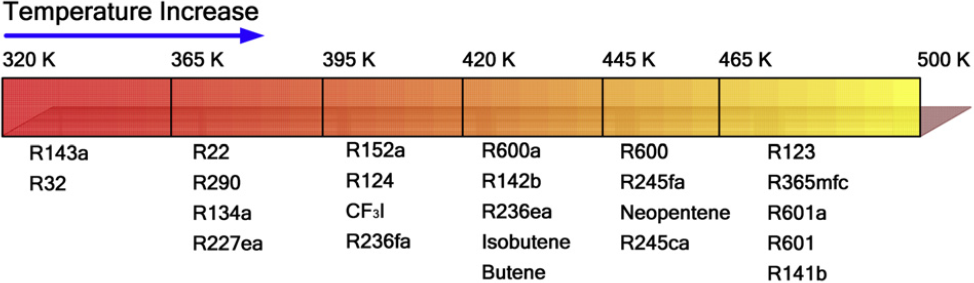
Figure 5. Recommended working fluids for binary cycles depending on the heat source temperature
Besides choosing working fluids, it is fundamentally important to choose the technological scheme of the cycle and, certainly, the turbine. Leaving the turbine outside of this consideration due to the specifics of the issue, we will consider various ORC schemes. The most common is the conventional Rankine cycle with overheating, shown in Fig. 4(d). Various variations in the cycle are associated with taking into account the shape of the saturation curve [Fig. 4(a)–(c)], which mainly affects the value of overheating (DiPippo, 2005; Ogurechnikov, 2014). For temperatures of separated liquid above 170°C, using supercritical cycles is considered more suitable, especially for zeotropic mixtures (Alkhasov, 2008; Braimakis et al., 2015). Such a cycle is close to the so-called triangular cycle [see Fig. 4(e)], which is more efficient due to the minimal temperature difference between the coolant and the working fluid. For example, in Alkhasov (2008) it was shown that at a separation temperature of 180°C in a supercritical cycle with a pressure of Pe = 5.0 MPa, the turbine power increases by 11% compared to the subcritical cycle at Pe = 3.6 MPa. The density of the medium in front of the turbine increases by 1.7 times, which allows significantly reducing the dimensions of the equipment. However, a significant increase in pressure up to 45–50 bar compared to typical pressure values of 15–30 bar in subcritical cycles leads to huge technical difficulties.
Employing new substances with low critical parameters allows reaching supercritical conditions even for low temperatures of the separator. In the case of essentially overheated steam at the turbine outlet, especially for “dry” liquids, heat recovery-based cycles are already becoming standard (Alekseenko and Ogurechnikov, 2021; Franco, 2011). Here, the excess steam heat in front of the condenser, taken away using an economizer, is transferred to the liquid heat carrier in front of the evaporator. Such technological flow diagrams are used at binary stations in Guatemala (Zunil, 20 MW), Kenya (Oserian, 1.8 MW; Olkaria III, 13 MW), etc. Significantly more complex schemes, considered to be promising, are described in DiPippo (2005, 2015). The first of them is called the dual-pressure binary cycle which provides a two-stage heating/boiling process with low and high-pressure loops and, accordingly, two turbines. The second technological scheme is called the dual-fluid binary cycle where two interrelated Rankine cycles are used.
Below are several specific examples of commercial binary stations for which any data are known.
- A double-cycle GPP in the USA (Alkhasov, 2008) uses steam in the first cycle and isobutane – in the second cycle. Isobutane cycle parameters are as follows: the temperature of the separated liquid at the inlet is 167°C, at the outlet – 66°C; the temperature of saturated isobutane vapor at the turbine inlet – 125°C, at the outlet – 60°C; the flow rate of the separated liquid – 278 kg/s; the flow rate of isobutane – 45 kg/s; turbine power – 22.3 MW; cycle efficiency – 11.9%.
- Heber Binary Geothermal Demonstration Power Plant (DiPippo, 2005). In the early 1980s, the first station was built with a mixed coolant: 90% isobutane + 10% isopentane. A supercritical cycle at a pressure of 40 bar was implemented. At a separated liquid temperature of 182°C and a flow rate of 614 kg/s, the gross power reached 36 MW, but the net power was only 21 MW. Due to heavy losses, this station was shut down. In 1993, the second station was launched with 12 binary power units and a total capacity of 44 MW (31 MW net). Here, isopentane was used in the subcritical cycle. The separated liquid temperature was 165°C. The gross thermal efficiency reached 12.7%.
- Chena Geothermal Power Plant, Alaska (Aneke et al., 2011). The separated liquid temperature is 73.3°C, the flow rate – 33.4 kg/s. A subcritical Rankine cycle with a slightly overheated freon R134a has been implemented. The vapor pressure in front of the turbine is 16 bar, behind the turbine – 4.4 bar. Gross power amounts to 250 kW, net power – to 210 kW. The thermal efficiency is 8%.
Thus, the following conclusions can be drawn from the presented analysis.
- Binary cycles occupy a leading position in terms of growth rates among energy technologies (both in geothermal energy and other energy sectors) being among the most promising technologies for future energy, especially for distributed generation.
- The prevailing schemes of binary power units are based on the organic Rankine cycle (ORC) with several variations, including subcritical cycles with overheating, as well as supercritical cycles.
- There is a huge variety of potential working fluids (many dozens) for ORC but only a limited number of fluids are used for commercial purposes. Preference is given to so-called “isentropic” as well as “dry” working fluids.
- Due to many criteria for selecting working fluids and a wide range of conditions to be met, there are no universal working fluids like water in the heat power industry. In each specific case, it is necessary to perform multivariate optimization studies to make a suitable choice of working fluids, as well as technological schemes and operating modes.
REFERENCES
Alkhasov, A.B. (2008) Geothermal Energy. Problems, Resources, Technologies. Moskow: Fizmatlit, 375 p. (in Russian).
DiPippo, R. (2005) Geothermal Power Plants. Principles, Applications, and Case Studies, Elsevier, Oxford, England, 450 p.
The Future of Geothermal Energy in the 21st Century Impact of Enhanced Geothermal Systems (EGS) on the United States. Report. (2006), Massachusetts Institute of Technology. 358 p.
Bertani, R. (2012) Geothermal Power Generation in the World 2005–2010 Update Report, Geothermics, 4, pp. 1–29.
Ogurechnikov, L.A. (2014) Saving Energy Resources. Generation of Electric Energy and Heat Supply Based on Low-Potential Sources, Lap Lambert Academic Publishing, Germany, 130 p.
Bao, J. and Zhao, L.A. (2013) Review of Working Fluid and Expander Selections for Organic Rankine Cycle, Renewable and Sustainable Energy Reviews, 24, pp. 325–342.
Wang, D.X., Ling X., Peng H., Liu L., and Tao L.L. (2013) Efficiency and Optimal Performance Evaluation of Organic Rankine cycle for Low-Grade Waste Heat Power Generation, Energy, 50, pp. 343–352.
Alekseenko, S.V. and Ogurechnikov, L.A. (2019) Concerning Utilization of Heat From Low-Potential Sources, Thermal Science, 23, 6B, pp. 4023–4030.
Alekseenko, S.V. and Ogurechnikov, L.A. (2021) Heat Exchange in Steam Generator with Low-Boiling Point Fluid, Thermal Science, 25, 5A, pp. 3569–3578. DOI: 10.2298/TSCI200220204A.
Braimakis, K., Preißinger, M., Brüggemann, D., Karellas, S., and Panopoulos, K. (2015) Low-grade Waste Heat Recovery with Subcritical and Supercritical Organic Rankine Cycle Based on Natural Refrigerants and Their Binary Mixtures, Energy, pp. 1–13.
Franco, A. (2011) Power Production from a Moderate Temperature Geothermal Resource with Regenerative Organic Rankine Cycles, Energy for Sustainable Development, 15, pp. 411–419.
DiPippo, R. (2015) Geothermal Power Plants: Evolution and Performance Assessments, Geothermics, 53, pp. 291–307.
Aneke, M., Agnew, B., and Underwood, C. (2011) Performance analysis of the Chena binary geothermal power plant, Applied Thermal Engineering, 31, pp. 1825–1832.
Referências
- Alkhasov, A.B. (2008) Geothermal Energy. Problems, Resources, Technologies. Moskow: Fizmatlit, 375 p. (in Russian).
- DiPippo, R. (2005) Geothermal Power Plants. Principles, Applications, and Case Studies, Elsevier, Oxford, England, 450 p.
- The Future of Geothermal Energy in the 21st Century Impact of Enhanced Geothermal Systems (EGS) on the United States. Report. (2006), Massachusetts Institute of Technology. 358 p.
- Bertani, R. (2012) Geothermal Power Generation in the World 2005–2010 Update Report, Geothermics, 4, pp. 1–29.
- Ogurechnikov, L.A. (2014) Saving Energy Resources. Generation of Electric Energy and Heat Supply Based on Low-Potential Sources, Lap Lambert Academic Publishing, Germany, 130 p.
- Bao, J. and Zhao, L.A. (2013) Review of Working Fluid and Expander Selections for Organic Rankine Cycle, Renewable and Sustainable Energy Reviews, 24, pp. 325–342.
- Wang, D.X., Ling X., Peng H., Liu L., and Tao L.L. (2013) Efficiency and Optimal Performance Evaluation of Organic Rankine cycle for Low-Grade Waste Heat Power Generation, Energy, 50, pp. 343–352.
- Alekseenko, S.V. and Ogurechnikov, L.A. (2019) Concerning Utilization of Heat From Low-Potential Sources, Thermal Science, 23, 6B, pp. 4023–4030.
- Alekseenko, S.V. and Ogurechnikov, L.A. (2021) Heat Exchange in Steam Generator with Low-Boiling Point Fluid, Thermal Science, 25, 5A, pp. 3569–3578. DOI: 10.2298/TSCI200220204A.
- Braimakis, K., Preißinger, M., Brüggemann, D., Karellas, S., and Panopoulos, K. (2015) Low-grade Waste Heat Recovery with Subcritical and Supercritical Organic Rankine Cycle Based on Natural Refrigerants and Their Binary Mixtures, Energy, pp. 1–13.
- Franco, A. (2011) Power Production from a Moderate Temperature Geothermal Resource with Regenerative Organic Rankine Cycles, Energy for Sustainable Development, 15, pp. 411–419.
- DiPippo, R. (2015) Geothermal Power Plants: Evolution and Performance Assessments, Geothermics, 53, pp. 291–307.
- Aneke, M., Agnew, B., and Underwood, C. (2011) Performance analysis of the Chena binary geothermal power plant, Applied Thermal Engineering, 31, pp. 1825–1832.